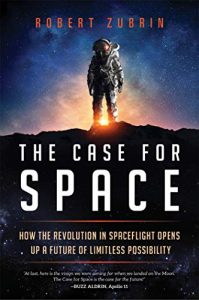
Author: Robert Zubrin
Rating: 10/10
An updated edition of a brilliant book “Entering Space” from the author of “The Case for Mars” that inspired Elon Musk’s ambition to settle the Red Planet.
Part 1 of the book shows how we can conquer space; part 2 gives reasons why we must. The Case for Space offers insights into what awaits us near Jupiter and Saturn, the strengths and weaknesses of SpaceX, and why we need a new frontier to drive innovation and prevent cultural and intellectual stagnation.
Incredibly well written and enlightening, this is an absolute must-read for all space enthusiasts.
Highlights
Lockheed Martin CEO Norm Augustine, declared that NASA’s moon program had to be canceled because the development of the necessary heavy-lift booster would take twelve years and $36 billion. Yet SpaceX did it, in half the time and at a thirtieth of the cost. And, to cap it all, the launch vehicle is three-quarters reusable. This is a revolution. The moon is now within reach. Mars is now within reach.
A reusable rocket system that can send 60 or 150 tons to orbit can also send 60 or 150 tons from New York to Sydney in less than an hour. For comparison, a Boeing 737 has an empty weight of 45 tons. If the Falcon Heavy or its successor, the Starship two-stage launch system, can be made fully reusable, then an entirely new market for space launch can be created, one involving not a hundred or so launches per year, as is currently the case, but hundreds or even thousands per day. Such a market would drive a radical cheapening of space technology, finally making possible all the dreams of space tourism, industrialization, and colonization that have been within view but out of reach since the dawn of the space age.
SpaceX has shown that it is possible for lean, hard-driving entrepreneurial ventures to do—better—what it previously was thought only the governments of major powers could attempt. With the naysayers refuted, dozens of would-be emulators from around the world are sure to enter the fray, and their fierce competition will crash the cost of space launch and, with it, the cost of developing more advanced in-space technology as well.
Russian space visionary Nikolai Kardashev outlined a schema for classifying civilizations.1 According to Kardashev, a Type I civilization was one that had achieved full mastery of all the resources of its planet. A Type II civilization was one that had mastered its solar system, while a Type III civilization would be one that had control of the full potential of its galaxy.
There is no law of physics that requires spaceflight to be hopelessly expensive. On the contrary, the energy per unit mass that must be added to an object to send it from the ground to low Earth orbit is about nine kilowatt-hours per kilogram. At a typical American price of $0.08 per kilowatt-hour, this would amount to a cost of $72 to send an eighty-kilogram person with twenty kilograms of luggage to the space station.
A Boeing 747 seats four hundred people and costs $400 million. If the plane were expended after every flight, the ticket price for a round-trip would have to be at least $2 million each,
Where there’s a will, there’s a way. Where there isn’t a will, there isn’t a way.
The central institutional impediment to space progress is the system of cost-plus contracting the government has put into place in the very foolish belief that the price of hardware could best be kept under control by regulating contractors to charge their documented costs plus a modest set profit rate (of perhaps 8–10 percent).
In the free enterprise world, manufacturers increase profit by cutting costs. In the cost-plus contractor world, manufacturers increase profit by increasing costs.
..the inflation of the cost of space launch leads to the inflation of the cost of everything else associated with spaceflight. For example, if you are planning a communications satellite business and the cost of your launch to orbit is $100 million, it really doesn’t matter much whether the cost of your satellite is $1 million or $20 million, because the difference only adds 20 percent to the capital cost of your operation overall. In fact, it probably drives your satellite cost over $50 million, because if you are paying $100 million just to get the satellite to its operating orbit, it damn well better work. So you are going to be more than willing to pay Cadillac prices for all your satellite’s components and spare no expense in testing everything to the max. Moreover, if the satellite costs $50 million, the launch vehicle damn well better work too, so its cost will be sent up further as well, driving the cost spiral even higher. Furthermore, since neither the spacecraft nor the launch vehicle can afford to risk failure, only components that have previously been proven in spaceflight will generally be acceptable for use, a catch-22 that has led to colossal technological stagnation.
How cheap can spaceflight get? As noted above, the energy to reach orbit is about the same as a round-trip flight from Los Angeles to Sydney: around $2,000 per passenger, or $20 per kilogram, including luggage. Air travel took a while before it could obtain such economies, so as a near-term goal for spaceflight, we’ll estimate ten times that cost, or $200/kg to launch to low Earth orbit. To go to someplace interesting beyond low Earth orbit, we’ll assume a cost ten times greater still, so $2,000/kg to go to the moon or Mars. As a check on these numbers, we note that it only takes about twenty-five kilograms of propellant to send one kilogram to orbit, and rocket propellant (for example, current kerosene/oxygen bipropellant—methane/oxygen would be cheaper) has a cost on the order of $0.40/kg. So the propellant cost of orbital launch is only about $10/kg—a figure that strongly supports the attainability of a $200/kg orbital delivery price for a reusable launch vehicle, with plenty of room for future improvement.
Beyond Mars lies the asteroid belt, containing vast amounts of platinum-group metal resources with values of more than $40,000 per kilogram, making them exploitable at $200/kg launch costs. Their exploitation will make them much cheaper, to be sure, but will cheapen launch and deep space transportation costs as well—and, more to the point, make important new technologies such as fuel cells (whose expense is driven by platinum prices) economical. This will have enormous benefits for life on Earth.
Beyond the asteroids are the outer planets, whose atmospheres contain virtually unlimited resources of helium-3, the fuel for fusion reactors that can give humanity an endless supply of pollution-free energy and the means to take us to the stars.
A general rule in aerospace is that cost of hardware increases in proportion to the square root of its size.
Kleos—eternal glory for doing great deeds.
SpaceX’s Falcon Heavy employs a triplet of the same kerosene/oxygen first stages that power its veteran Falcon 9 medium lifter. In contrast, New Glenn is a totally new system that will use a single large-core methane/oxygen booster for takeoff. But while the designs of the two systems are completely different, their fifty- to sixty-ton-to-orbit delivery capacities (about twice that of the much more expensive Atlas and Delta systems offered by the Lockheed Martin–Boeing United Launch Alliance) are close to identical, making them direct competitors. So the new space race is on.
The key reusable commercial competitors for the near term are the SpaceX Falcons and the Blue Origin New Glenn series. The United Launch Alliance’s veteran Atlas and Delta series are being priced out of the market, a trend ULA hopes to correct with its new Vulcan booster. The Russians and French are going to have to come up with something better than their expendable Soyuz, Proton, and Ariane rockets soon if they want to remain competitive. The NASA SLS offers almost twice the lift capability of the Falcon Heavy or New Glenn, but its costs are an order of magnitude greater. The Saturn V moon rocket could lift even more (140 tons to orbit) but went out of production almost half a century ago. At the small end, there is a new generation of entrepreneurial microsatellite launchers, typified by the Vector-R and the Electron-sized Vector-H.
Even with full reusability, if we want to get the cost per launch down by a factor of a hundred, we are going to need a market for at least a hundred times as many launches.
The key to global transport using rocket propulsion is a two-stage, fully reusable system in which the first stage returns to the launch site and the second makes the long-distance trip, to be sent home with the help of a first stage based at the destination.
To obtain global reach, a rocket must reach orbital velocity, which in the case of the Earth is eight kilometers per second. However, a rocket experiences velocity loss during ascent due to aerodynamic drag and gravity, and so the real velocity increment (or “delta-V,” abbreviated ∆V) that needs to be delivered by its propulsion system is closer to 9.5 km/s. This is more than twice the exhaust velocity of any practical propellant combination, which means that the amount of propellant needed will greatly outweigh the payload.
By my math, each flight of a one-hundred-ton passenger cabin rocket plane would consume about 2,100 tons of methane/oxygen propellant. At a cost of $120 per ton, this would entail a fuel bill of about $250,000 per trip. At 140 tons, including cabin, tanks, and engines, the long-distance flight vehicle would have a dry mass comparable to a Boeing 767, which carries about two hundred passengers. If each passenger paid the $20,000 price of current global-distance first-class tickets (getting, in addition to fast global transportation, ten times the Virgin Galactic zero-gravity fun for one-tenth the price), a gross revenue of $4 million per flight could be obtained. That leaves plenty of room for other operating costs besides propellant, even allowing for some tickets to go for less than the premium price.
the famous rocket equation, which says that for any rocket with exhaust velocity C, the “mass ratio,” the ratio of its total mass (i.e., its dry mass plus propellant) to its dry mass alone increases exponentially with the factor (∆V/C). Put mathematically:
On orbit, the distorting influence of gravity is nearly absent, which creates conditions enabling the production and determination of the structure of various types of crystals and other compounds. In addition, low Earth orbit provides access to very high-quality vacuum conditions that cannot be economically produced in earthbound labs.
Potential microgravity or high-vacuum research products with astronomical value exist in the form of vaccines, synthetic collagen (which could be used to construct corneas), targetable pharmaceuticals, structured proteins, crystal materials (for computer chips and quantum devices), ultrapure epitaxial film production, unique polymers and alloys, and electrophoresis applications. These products could lead to breakthrough applications in such high-growth areas as semiconductors, computers, instruments, biotechnology, and drug manufacturing, areas that today represent a business base of more than $240 billion per year.
Producing patents is the best way for an orbiting lab to make money. The discovery of knowledge in space that enables industrial processes to be realized on Earth is clearly the highest payoff path for such space-based facilities.
Finally, the sales volume of the product must be sufficiently high. If the basic costs of running the orbital lab are $40 million per year (a number that would allow shipping about one hundred tons per year of various supplies, assuming half the budget was so dedicated), the operation will still fail if only twenty tons of its $2,000/kg wholesale-priced product can be sold. To allow for all the business’s costs, at least $80 million in gross revenue would be needed, or forty tons of product. Let’s say that the end use for the product was a drug or computer chip selling retail for $200 for a hundred-gram unit. In that case, four hundred thousand units would have to be sold per year. Given a sufficiently desirable and unique product, sales numbers such as these are entirely feasible.
In 2000, the world’s total global data traffic was four thousand terabytes (TB) per month. It is now (2019) approaching forty million TB/month and by 2030 is projected to reach four billion TB/month.
Current communication satellites operate in geosynchronous orbit, thirty-six thousand kilometers above the surface of the Earth. At this height, they orbit the Earth at exactly the same rate that it turns, thereby keeping themselves in the same place in the sky as seen from the ground. This feature, as the concept’s inventor, science fiction writer Arthur C. Clarke, realized, is extremely convenient for communications purposes, as it allows ground-based antennas to be fixed in their aim while permitting nearly the entire Earth to be covered by just three satellites spaced 120 degrees apart around the planet’s equatorial plane. But to transmit from such a distance, the satellites need to be large and power-hungry, and thus expensive. Moreover, it takes about 0.25 seconds for radio signals to make the seventy-two-thousand-kilometer round-trip from the Earth’s surface up to geosynchronous orbit and down again. While this does not matter for applications like one-way radio or TV broadcasting, it poses serious problems for two-way communication. For long-distance telephone calls, the quarter-second signal time delays each way can be quite annoying. For systems attempting to remotely control machinery under dynamic conditions (for example, self-driving cars or aircraft), it could potentially be catastrophic.
If the satellites could orbit lower, say 1,200 kilometers, the time delay could be cut thirtyfold and the transmission power each way reduced a thousandfold. But satellites at that height orbit the Earth every two hours and can only be seen from the ground when they are relatively close by. As a result, they are constantly moving in and out of view, with each one only providing temporary coverage to a limited ground area as it travels along its orbital track. If you want to provide global communication coverage using spacecraft at such altitudes, you can’t do it with three satellites; you will need thousands of them.
By February 2017, OneWeb announced that it had already sold out the capacity of its 648 satellites—before a single one had been launched or even built. So, raising another billion dollars from the SoftBank Group, the company expanded its planned constellation to 2,420 satellites.7 Shortly thereafter, Samsung proclaimed it had plans for a Space Internet altitude constellation consisting of 4,600 satellites, orbiting at 1,557 kilometers altitude.8 Not to be outdone, in May 2017, Elon Musk announced that his SpaceX company would be fielding its own constellation, named Starlink, consisting of 4,425 small satellites orbiting at 1,200 kilometers, operating in eighty-three orbital planes. In turn, these crafts would be supplemented with a further 7,518 satellites flying at just 340 kilometers altitude.
At $5,000 per kilogram, a ten-thousand-kilogram satellite would cost $50 million to launch, but a ten-kilogram unit only $50,000. If you want to get into space on the cheap, this could be the easiest way to do it. Such is the approach taken by professors Robert Twigg (of Stanford University) and Jordi Puig-Suari (of Cal Poly, San Luis Obispo), the inventors of the CubeSat. By the late 1990s, it had become clear to these two that it should be possible to build a minimal-capability satellite with a mass of one kilogram and a volume of one liter, or a cube about four inches on a side.
A favorite configuration is the 6U version, with dimensions of ten by twenty by thirty centimeters, or about the size of a shoebox.
Once the space launch revolution is factored in, the cost of sending a highly capable ten-kilogram 6U CubeSat could drop from $50,000 to as low as $2,000, making space exploration an affordable activity not just to government, corporations, universities, and billionaires but even to private individuals of middle-class means.
In space, solar energy is available twenty-four hours a day, not masked by the dulling effect of the Earth’s atmosphere. Moreover, while most terrestrial solar arrays are fixed in orientation, an orbital solar array can track the sun. Avoiding the atmosphere increases the effective solar brightness by a factor of about 1.5, while the ability to track the sun multiplies the average power produced by the orbiting array by a factor of four. Thus, when both advantages are considered, an orbital solar array can produce a time-averaged output about six times greater per unit area than its counterpart fixed in orientation on the ground in an equatorial desert. The SPS unit beams its solar-produced power via microwaves to Earth, where it is received by a “rectenna.” The microwave energy is then converted to high-voltage alternating power for consumption on the consumer grid. About half the power would be lost in the beaming process, reducing the orbital array’s advantage over its terrestrial counterpart to a factor of three. However, as a countervailing advantage, the groundside rectenna is smaller than a solar array, and cheaper, and can be put nearly anywhere in the world, including places where solar power is frequently unavailable due to weather.
The cost of delivering payloads to GEO, however, is about four times that of LEO, running in the range of $20,000/kg today, and would still be $800/kg after the space launch revolution. Therefore, the launch cost of the SPS would be about $12,800/kW or $12.8 billion for a one-thousand-megawatt unit suitable for providing the power needs of a city the size of Denver.
Space superiority means having better space assets than an opponent. Space supremacy means being able to assert a complete monopoly of such capabilities.
Here’s how the mission plan could work. The Falcon Heavy can lift sixty tons to low Earth orbit (LEO). Starting from that point, a hydrogen/oxygen rocket-propelled cargo lander could deliver ten tons of payload to the lunar surface. We, therefore, proceed by sending two such landers to our planned base location. The best place for it would be at one of the poles because there are spots at both of the moon’s poles where sunlight is accessible all the time, as well as permanently shadowed craters nearby where water ice has accumulated. Such ice could be electrolyzed to make hydrogen/oxygen rocket propellant to fuel both Earth Return Vehicles as well as flying rocket vehicles that would provide the base’s crew with exploratory access to most of the rest of the moon.
The lunar surface contains vast amounts of oxygen, silicon, iron, titanium, magnesium, calcium, and aluminum, tightly bound into rocks as oxides, but there nevertheless.
The moon also possesses scarce, but in principle obtainable, supplies of helium-3, an isotope otherwise naturally nonexistent in the inner solar system. Helium-3 offers a number of potentially important advantages as a fuel for thermonuclear fusion reactors and thus perhaps could provide a future lunar colony with a cash export commodity.
while the moon’s rocks and soils possess ample supplies of oxygen and several important metals, they are entirely lacking in such vital substances as organics, hydrates, carbonates, nitrates, sulfates, phosphates, and salts. The key primary biogenic elements of hydrogen, carbon, and nitrogen are present on the moon, but in general only in extremely rare quantities (about fifty parts per million) in surface materials impregnated by the solar wind.
the leading secondary biogenic elements, sulfur, and phosphorus are also on the rare side, with typical concentrations in lunar soils ranging from five hundred to one thousand parts per million. Among the leading secondary industrial elements, potassium, manganese, and chromium are reasonably common (about 2,000 ppm), but nickel and cobalt are rather scarce (about 200 ppm and 30 ppm, respectively, in typical regolith, though they may be more accessible in certain places as meteor impact debris), while copper, zinc, lead, fluorine, and chlorine are extremely hard to come by (5–10 ppm each). Helium is present in solar-wind-impregnated regolith in concentrations of about 10 ppm, while argon and neon can be found in concentrations of about 1 ppm each.
The Apollo-era LEM ascent vehicle had a dry mass of two metric tons.6 The SpaceX Dragon capsule, which can carry crew and has a heat shield capable of withstanding reentry from Mars return (which is more demanding than the moon), has a mass of eight metric tons. The velocity increment ∆V required to fly directly back to Earth from the lunar surface and do a direct entry into the Earth’s atmosphere is three kilometers per second. If a spacecraft is using hydrogen/oxygen rocket propulsion, which has an exhaust velocity of 4.5 km/s, it will require as much propellant as its own dry mass to execute such a maneuver (meaning that in technical terms, it would have a “mass ratio” of two, because its wet mass is twice its dry mass.)
In order to deliver twenty tons to the lunar surface, we would need to send forty tons to lunar orbit. As it happens, the 4.2 km/s ∆V required to send a spacecraft from LEO to lunar orbit is exactly the same as that needed to go from Earth orbit to Mars on a six-month transit trajectory. So, the same system that can send forty tons to lunar orbit can send an equal cargo on its way to Mars—which is enough to do the job.
Rocket Lab’s Electron launch vehicle takes flight from its New Zealand launchpad for the first time on May 25, 2017. The flight reached space but failed to make orbit. But on its second launch on January 23, 2018, Electron made it all the way, successfully delivering two Spire Lemur-2 CubeSats to their desired circular orbit. Electron has a payload capacity of two hundred kilograms, making it an excellent choice to launch constellations of microsatellites.